This paper investigates cellulose’s molecular structure, chemical characteristics, and reactivity, focusing on its wide range of industrial applications, including textile manufacture, new biodegradable materials, and biofuels.
Clik here to view.
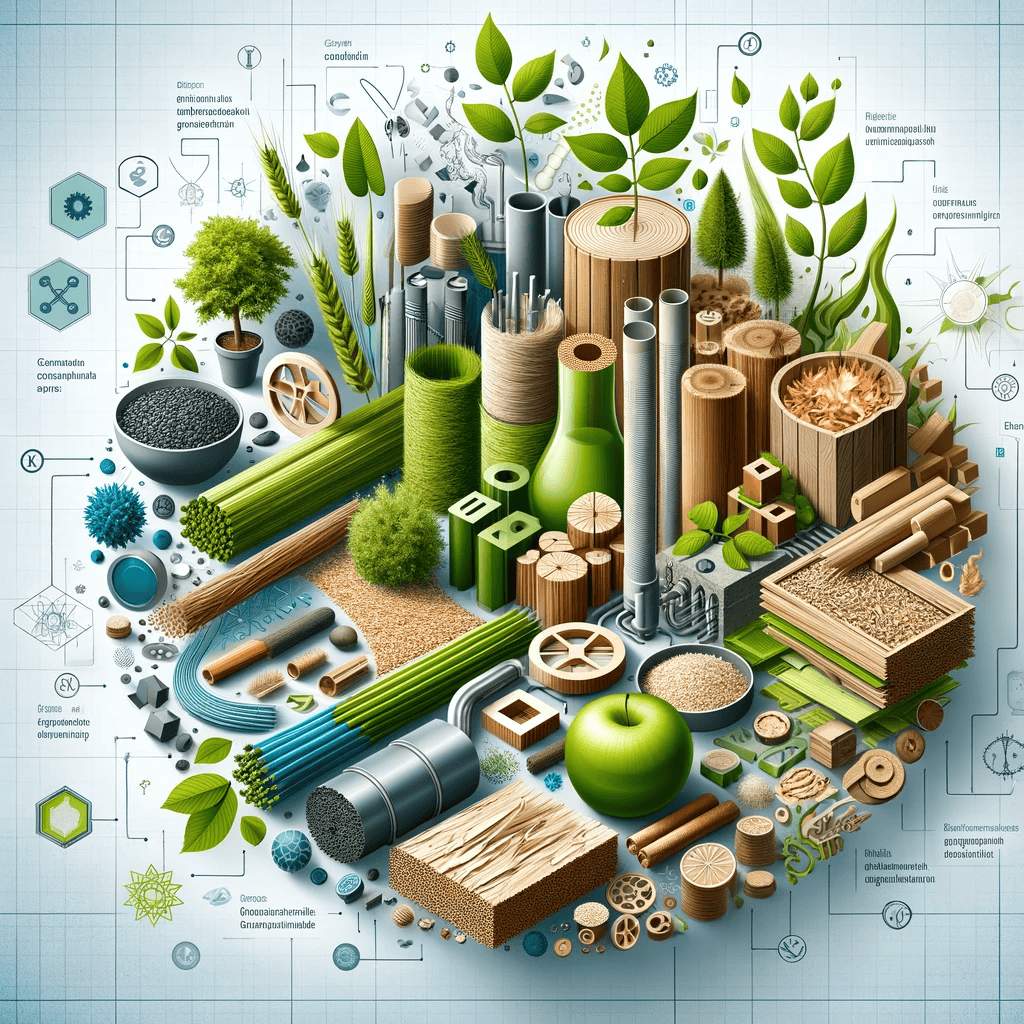
Introduction to Cellulose
Cellulose is a fundamental natural polymer with important biological properties and a vast distribution in nature. It plays an important role in plant cell walls by providing structural support and rigidity. Cellulose’s molecular structure consists of long chains of glucose units connected by β(1→4) glycosidic linkages. This arrangement creates crystalline regions known as cellulose crystallites, which are bound together by Van der Waals interactions and hydrogen bonding (Leppänen et al., 2009).
Cellulose can come from a variety of sources, including plants, microbes, and recycled items such as newspapers. Sugars such as sucrose and glucose produce high yields of bacterial cellulose (Mikkelsen et al., 2009). Cellulose derivatives, including methyl cellulose and carboxymethylcellulose, are also used to create functional materials such as hydrogels for tissue engineering applications (Dutta et al., 2019).
The adaptability of cellulose extends to its nanoscale forms, such as nanocrystalline cellulose (NCC) and nanofibrillated cellulose (NFC), which have high aspect ratios and huge surface areas, making them useful in a variety of advanced applications (Fatema et al., 2022). Furthermore, cellulose nanoparticles can be separated using a variety of methods, including chemical, mechanical, and biological processes, yielding materials such as microcrystalline cellulose and bacterial cellulose (Varqani, 2023).
Cellulose is a remarkable biopolymer and sustainable raw material with numerous uses in medicine, nanotechnology, and materials science. Its structural diversity, abundance in nature, and biocompatibility make it an excellent resource for the development of novel biomaterials and functional products.
Chemical Properties and Reactivity
Cellulose has several important chemical characteristics that affect its behavior and reactivity. One important characteristic is crystallinity, which relates to the arrangement of cellulose chains in crystalline and amorphous regions. The degree of crystallinity influences cellulose’s enzymatic digestibility, with higher crystallinity inhibiting the process (Mittal et al., 2011). Furthermore, cellulose can exist in several crystal forms, including cellulose I and cellulose II, which can influence its properties and applications (Singh et al., 2019).
Cellulose’s solubility is limited by its strong intermolecular hydrogen bonding network. However, cellulose can be dissolved in particular solvents such as ionic liquids, resulting in cellulose regeneration with changed characteristics (Taheri et al. 2019). Furthermore, treatments like alkaline or liquid-ammonia processing can affect cellulose’s crystalline structure and enzymatic digestibility (Mittal et al., 2011).
Cellulose undergoes several chemical processes, including hydrolysis and esterification. Hydrolysis is the breaking of glycosidic bonds between glucose units, which results in the degradation of cellulose into smaller fragments. The hydrolysis behavior of cellulose varies between amorphous and crystalline areas, which influences reaction kinetics (Yu & Wu, 2010). Esterification procedures involve adding ester groups to cellulose molecules, modifying their characteristics for specific uses (Karst et al., 2006).
The chemical characteristics of cellulose, such as crystallinity, solubility, and reactivity, have a substantial impact on its behavior and prospective applications. Understanding these qualities is critical for properly exploiting cellulose in a variety of sectors, including materials research and biofuel generation.
Applications of Cellulose
Cellulose, a versatile biopolymer, has many applications in numerous industries, demonstrating its versatility and sustainability. Because of its unique structural properties and renewable nature, cellulose is an important raw material in the textile industry (Felgueiras et al., 2021). Recent breakthroughs have resulted in the development of ecologically friendly cellulose fiber technologies and bacterial cellulose biomaterials, broadening the range of textile applications (Klemm et al. 2005). Additionally, cellulose-based textiles have been investigated for their antibacterial qualities, which improve comfort, durability, and health (Emam, 2019).
Cellulose is an important fundamental component in paper manufacture, helping to produce wood-based regenerated cellulose fibers and enabling the production of high-quality paper products (Björquist et al., 2017). Furthermore, advances in plasma treatment have been used to improve the oleophobic characteristics of paper, creating new opportunities for its use (Resnik et al., 2021).
With the introduction of cellulose-based polymers, bioplastics have advanced significantly. Cellulose fibers are mixed into thermoplastic composite materials, providing environmentally friendly alternatives for injection molding and extrusion processing (Immonen et al., 2020). The development of cellulose-derived hydrogels and electrospun fibers has led to eco-friendly textiles with enhanced characteristics for applications such as wound dressing (Jirawitchalert et al., 2022; Kamiński et al., 2020).
In the field of biofuels and biodegradable materials, cellulose stands out as a renewable and plentiful resource. Lignocellulosic biomass, largely consisting of cellulose, hemicellulose, and lignin, is an important feedstock for biofuel generation (Chen et al., 2021). Continuous attempts are being conducted to create sustainable cellulose filaments from sources such as paper sludge nanofibers, highlighting novel techniques to meeting the growing demand for regenerative cellulose fibers (Adu et al., 2021).
Overall, cellulose’s numerous applications in textiles, paper manufacture, bioplastics, biofuels, and biodegradable materials highlight its importance as a sustainable and multifunctional biopolymer with enormous potential across multiple industries.
References
Dutta, S., Patel, D., & Lim, K. (2019). Functional cellulose-based hydrogels as extracellular matrices for tissue engineering. Journal of Biological Engineering, 13(1). https://doi.org/10.1186/s13036-019-0177-0
Fatema, N., Ceballos, R., & Fan, C. (2022). Modifications of cellulose-based biomaterials for biomedical applications. Frontiers in Bioengineering and Biotechnology, 10. https://doi.org/10.3389/fbioe.2022.993711
Leppänen, K., Andersson, S., Torkkeli, M., Knaapila, M., Kotelnikova, N., & Serimaa, R. (2009). Structure of cellulose and microcrystalline cellulose from various wood species, cotton and flax studied by x-ray scattering. Cellulose, 16(6), 999-1015. https://doi.org/10.1007/s10570-009-9298-9
Mikkelsen, D., Flanagan, B., Dykes, G., & Gidley, M. (2009). Influence of different carbon sources on bacterial cellulose production bygluconacetobacter xylinusstrain atcc 53524. Journal of Applied Microbiology, 107(2), 576-583. https://doi.org/10.1111/j.1365-2672.2009.04226.x
Varqani, N. (2023). Review: utilization of cellulose in food products. Iop Conference Series Earth and Environmental Science, 1230(1), 012039. https://doi.org/10.1088/1755-1315/1230/1/012039
Karst, D., Yang, Y., & Tanaka, G. (2006). An explanation of increased hydrolysis of the β-(1,4)-glycosidic linkages of grafted cellulose using molecular modeling. Polymer, 47(18), 6464-6471. https://doi.org/10.1016/j.polymer.2006.07.006
Mittal, A., Katahira, R., Himmel, M., & Johnson, D. (2011). Effects of alkaline or liquid-ammonia treatment on crystalline cellulose: changes in crystalline structure and effects on enzymatic digestibility. Biotechnology for Biofuels, 4(1). https://doi.org/10.1186/1754-6834-4-41
Singh, A., Ranawat, B., & Meena, R. (2019). Extraction and characterization of cellulose from halophytes: next generation source of cellulose fibre. Sn Applied Sciences, 1(11). https://doi.org/10.1007/s42452-019-1160-6
Taheri, N., Abdolmaleki, A., & Fashandi, H. (2019). Impact of non‐solvent on regeneration of cellulose dissolved in 1‐(carboxymethyl)pyridinium chloride ionic liquid. Polymer International, 68(12), 1945-1951. https://doi.org/10.1002/pi.5903
Yu, Y. and Wu, H. (2010). Significant differences in the hydrolysis behavior of amorphous and crystalline portions within microcrystalline cellulose in hot-compressed water. Industrial & Engineering Chemistry Research, 49(8), 3902-3909. https://doi.org/10.1021/ie901925g
Adu, C., Zhu, C., Jolly, M., Richardson, R., & Eichhorn, S. (2021). Continuous and sustainable cellulose filaments from ionic liquid dissolved paper sludge nanofibres. Journal of Cleaner Production, 280, 124503. https://doi.org/10.1016/j.jclepro.2020.124503
Björquist, S., Aronsson, J., Henriksson, G., & Persson, A. (2017). Textile qualities of regenerated cellulose fibers from cotton waste pulp. Textile Research Journal, 88(21), 2485-2492. https://doi.org/10.1177/0040517517723021
Chen, Y., Wang, W., Liu, P., Lin, A., Fan, X., Wu, C., … & Wei, D. (2021). The novel repressor rce2 competes with ace3 to regulate cellulase gene expression in the filamentous fungus trichoderma reesei. Molecular Microbiology, 116(5), 1298-1314. https://doi.org/10.1111/mmi.14825
Emam, H. (2019). Antimicrobial cellulosic textiles based on organic compounds. 3 Biotech, 9(1). https://doi.org/10.1007/s13205-018-1562-y
Felgueiras, C., Azóia, N., Gonçalves, C., Gama, M., & Dourado, F. (2021). Trends on the cellulose-based textiles: raw materials and technologies. Frontiers in Bioengineering and Biotechnology, 9. https://doi.org/10.3389/fbioe.2021.608826
Immonen, K., Jetsu, P., Keränen, J., & Torvinen, K. (2020). Feasibility of foam forming technology for producing wood plastic composites. Journal of Applied Polymer Science, 137(45). https://doi.org/10.1002/app.49404
Jirawitchalert, S., Mitaim, S., Chen, C., & Patikarnmonthon, N. (2022). Cotton cellulose-derived hydrogel and electrospun fiber as alternative material for wound dressing application. International Journal of Biomaterials, 2022, 1-12. https://doi.org/10.1155/2022/2502658
Kamiński, K., Jarosz, M., Grudzień, J., Pawlik, J., Zastawnik, F., Pandyra, P., … & Kołodziejczyk, A. (2020). Hydrogel bacterial cellulose: a path to improved materials for new eco-friendly textiles. Cellulose, 27(9), 5353-5365. https://doi.org/10.1007/s10570-020-03128-3
Klemm, D., Heublein, B., Fink, H., & Böhn, A. (2005). Cellulose: fascinating biopolymer and sustainable raw material. Angewandte Chemie, 44(22), 3358-3393. https://doi.org/10.1002/anie.200460587
Resnik, M., Levičnik, E., Gosar, Ž., Zaplotnik, R., Kovač, J., Ekar, J., … & Junkar, I. (2021). The oleofobization of paper via plasma treatment. Polymers, 13(13), 2148. https://doi.org/10.3390/polym13132148
The post Cellulose: Structure, Reactivity, and Innovative Industrial Applications first appeared on ChemH.